What is a PN junction?
PN junction diode acts as a valve. It allows current flow when Anode is at a higher potential than the cathode (i.e., forward bias). Conversely, it acts as an open circuit (very high resistance) when Anode is at a lower potential than the cathode (i.e., reverse bias).
What is anode and cathode of a diode?
In a PN junction diode, the terms “anode” and “cathode” refer to the two terminals of the diode. The PN junction combines a P-type semiconductor (with positively charged holes as majority carriers) and an N-type semiconductor (with negatively charged electrons as majority carriers).
- Anode: The anode is the diode’s positive (+) terminal. In a PN junction diode, the P-type semiconductor is associated with the anode. It is where the holes, which are positive charge carriers, are prevalent.
- Cathode: The cathode is the diode’s negative (-) terminal. In a PN junction diode, the N-type semiconductor is associated with the cathode. It is where the electrons, which are negative charge carriers, are prevalent.
The behavior of a PN junction diode is such that current can flow easily from the P-type region (anode) to the N-type region (cathode). If a positive voltage is applied at the anode relative to the cathode, the diode will allow current to flow and behave as a short circuit. This condition of having higher potential at the anode than the cathode is called the forward biased condition.
It restricts the current flow in the reverse direction when the anode potential exceeds the cathode potential. This condition is called the reverse biased condition. This property makes PN junction diodes useful for rectification and other electronic applications.
Construction of a PN junction
Usually, a p-type semiconductor is used to make a PN junction. One side of the p-substrate is diffused with 5-valent electron atoms (donors) to make the side N-type. The other side remains p-type because it was a p-substrate. Other ways of making diodes are ion-implantation of donor atoms into a p-substrate with depth control and epitaxy (growing) of donor atoms using chemical vapor deposition (CVD).
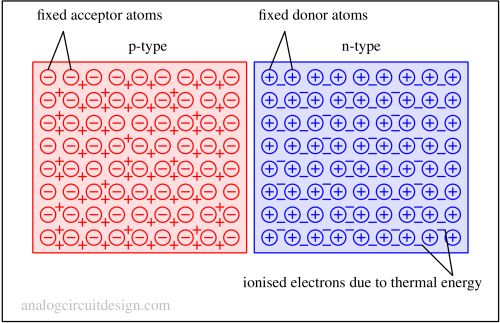
Formation of p-n junction
PN junction theory is a fundamental topic in circuit analysis. Understanding this topic will pave the way to explain BJT and MOS as well. It is ubiquitous in semiconductor based circuits.
As a thought experiment, let’s assume that the p-n junction is made by “joining” p-type and n-type material together. As soon as they are joined together, diffusion will happen from the area having high electrons/holes to the area having lesser electrons/holes. This is facilitated by the thermal energy of the electrons and holes. When the electrons from the n-side diffuse to the p-side, they leave back stationary positively charged donor atoms. These electrons fill the holes in the p-side and create stationary negatively charged acceptor atoms in the p-side. These stationary charges create an electric field that impedes diffusion. So, an equilibrium is reached. The electric field causes a drift current and the thermal energy causes the diffusion current. Both are present at any instant during equilibrium however they counter-balance each other.
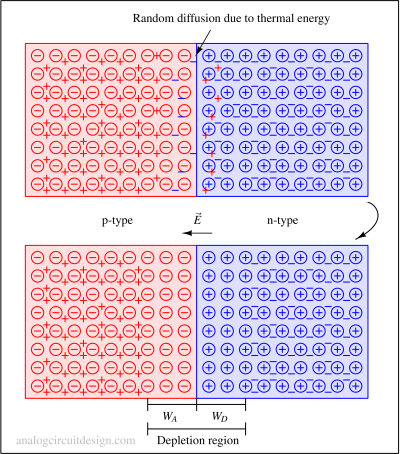
In no-bias conditions, electrons diffusing from the n-type region have to do positive work (against a force) to reach the p-type region due to the presence of the electric field. This positive work is nothing but a potential barrier (\(V_b\)). So, the main reason which is stopping this free thermal diffusion to happen is the electric field and the potential barrier related to it. For the thermal diffusion to happen continuously it is required to cancel or lower the electric field using an external bias. That is done using a forward bias. A forward bias (\(V_f\)) lowers the potential barrier and releases electrons like water from floodgates in a dam. Quantitative analysis about forward bias and reverse bias is done in PN junction theory – 2/2.
Energy band diagram of p-n junction
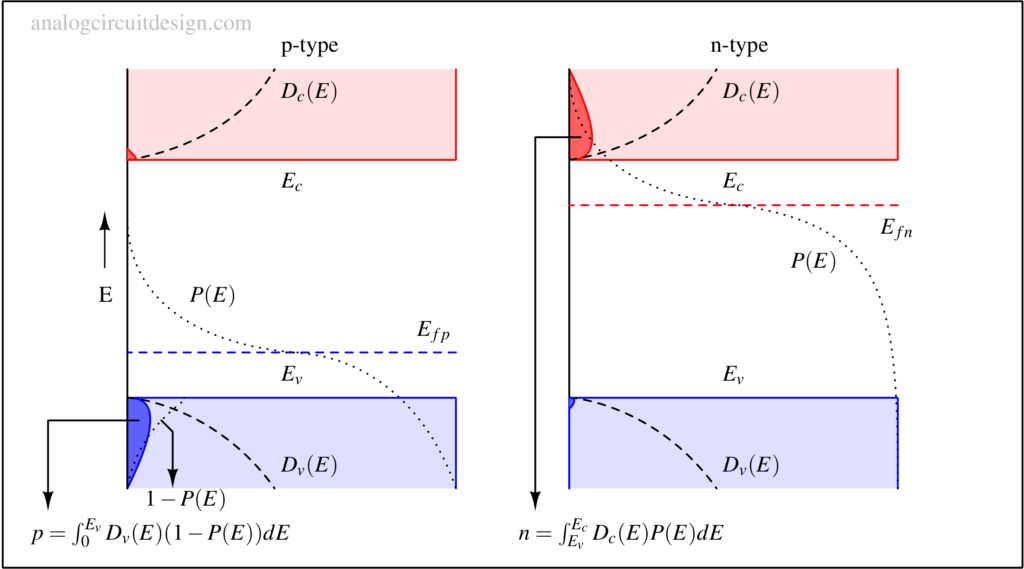
The band diagram is usually drawn in terms of electron energy. Due to thermal energy, some electrons spill above the Fermi level (\(E_f\)) and come to the conduction band. The same electron will jump back and forth randomly, but on average, some electrons will be above the conduction band due to thermal energy.
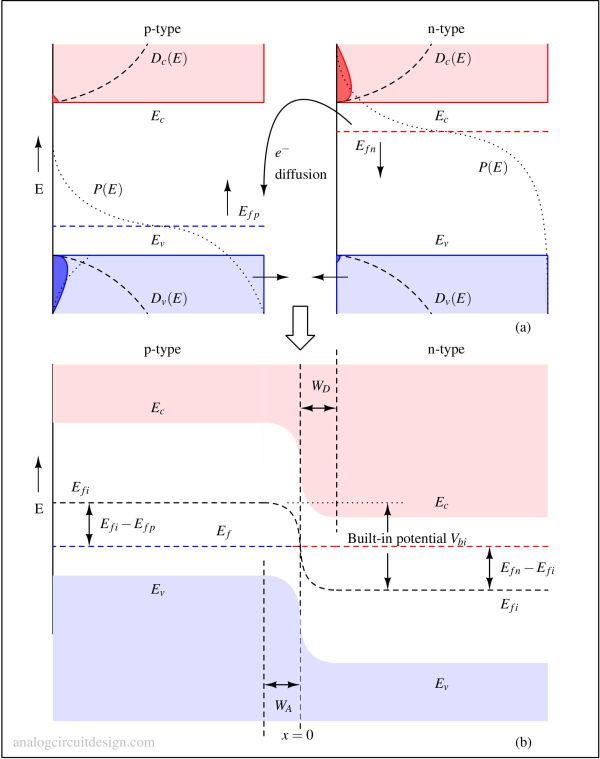
In equilibrium and with no external voltage bias, there is only one fermi level. This is because if there is a higher Fermi level, then due to thermal agitation, electrons will move from a higher Fermi level to a lower Fermi level. This will raise the level of lower Fermi level and bring down the level of higher Fermi level till both become equal as shown in Fig 4. In PN junction, fermi level of n-side is higher. So, electrons moved to region of lower fermi level (p-side) because of random thermal diffusion. This equalisation of Fermi level resulted in uncovering of positively charged atoms in n-side and negatively charged atoms in p-side causing an electric field as explained earlier. This electric field has now created a barrier resulting in energy band-bending.
How much is the built-in potential ?
In the sections above, we discussed the barrier potential (also called built-in potential) of the p-n junction. Now, the barrier potential will be derived using the difference of quasi-fermi levels and intrinsic Fermi levels.
From Fig. 4,
$$V_{bi} = (E_{fn} – E_{fi}) + (E_{fi} – E_{fp})$$
$$\implies{} V_{bi} = \cfrac{kT}{q}\ln{\left(\cfrac{N_D}{n_i}\right)}+\cfrac{kT}{q}\ln{\left(\cfrac{N_A}{n_i}\right)}$$
$$\implies{} V_{bi} = \cfrac{kT}{q}\ln{\left(\cfrac{N_AN_D}{n_i^2}\right)}$$
Depletion width of a p-n junction
Let’s assume that the equilibrium has reached and the depletion region is formed. Now, the built-in potential would be the energy required per charge to push the electrons from \(x = W_D\) to \(x = -W_A\). This pushing force is nothing but thermal agitation.
The electric field in the depletion region (\(-W_A<x<+W_D\)),
$$\vec{E} = -\cfrac{qN_D(W_D-x)}{\epsilon{}}, 0<x<W_D$$
$$\vec{E} = -\cfrac{qN_A(W_A+x)}{\epsilon{}}, -W_A<x<0$$
Electric field outside the depletion region will be 0, because there is 0 net charge enclosed by gaussian surface.
Work done \(W\) by thermal energy to push a electron from \(+W_D\) to \(-W_A\).
$$W = \int_{-W_A}^{W_D}(-q).\vec{E}.\vec{dx}$$
$$\therefore{} W = \cfrac{q^2}{2\epsilon{}}\left(N_AW_A^2+N_DW_D^2\right)$$
So, work done by a unit positive charge (also potential),
$$V_{bi} = \cfrac{W}{q} = \cfrac{q}{2\epsilon{}}\left(N_AW_A^2+N_DW_D^2\right)$$
Where,
$$q = +1.6\times{}10^{-19}\,\text{C}$$
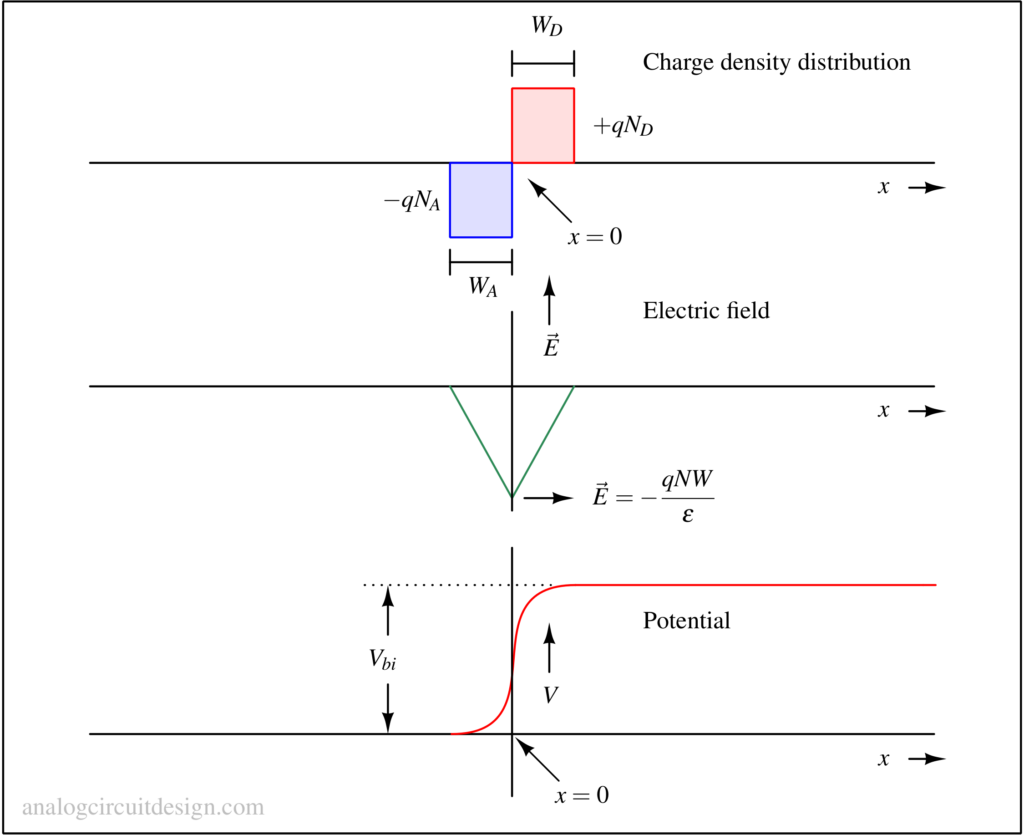
From charge neutrality in the depletion region,
$$N_DW_D = N_AW_A$$
Let’s assume, \(X_{dep} = W_A + W_D\). From the above equations,
$$V_{bi} = \cfrac{qX_{dep}^2}{2\epsilon{}}\cfrac{N_AN_D}{N_A+N_D}$$
$$X_{dep} = \sqrt{\cfrac{2\epsilon{}V_{bi}}{q}\left(\cfrac{1}{N_A}+\cfrac{1}{N_D}\right)}$$
From above, \(X_{dep}\) can be obtained by substituting \(V_{bi}\) from an earlier section.
This \(X_{dep}\) expression will help us to find the voltage-dependent capacitance formed by the depletion region. Notice the 2 opposite polarity charges are separated by \(X_{dep}\). Forward and Reverse biasing will modulate \(X_{dep}\). More about capacitance in P-N junction (2/2) .